
RETURN to Periodic Table
Oxygen is the 8th element on the periodic table because it has 8 protons in its nucleus. The protons will attract 8 electrons to surround the nucleus in order to form a neutral atom. With 8 protons and 8 neutrons, most oxygen atoms have an atomic mass of 16 amu.
Electron Shell
Oxygen has four electrons in its p-orbital. This is not a sphere-shaped harmonic, and so four p-electrons cannot achieve a stable electron symmetry around a spherical core. Oxygen therefore cannot simply add its four p-electrons on top of the same (2s2) configuration that beryllium has, as shown here, because it would not be a stable configuration:
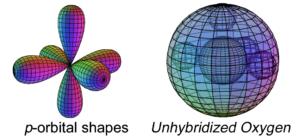
The asymmetry therefore causes oxygen to hybridize its 2s and 2p electrons in order to achieve tetrahedral symmetry. Its sp3 hybrid orbitals feature two di-electrons and 2 unpaired, degenerate electrons. This is why oxygen typically makes 2 bonds, and it is this tetrahedral geometry that gives the water (H2O) molecule its characteristic bent shape (see below). (The large wireframe below indicates the boundary of the n=2 shell.)
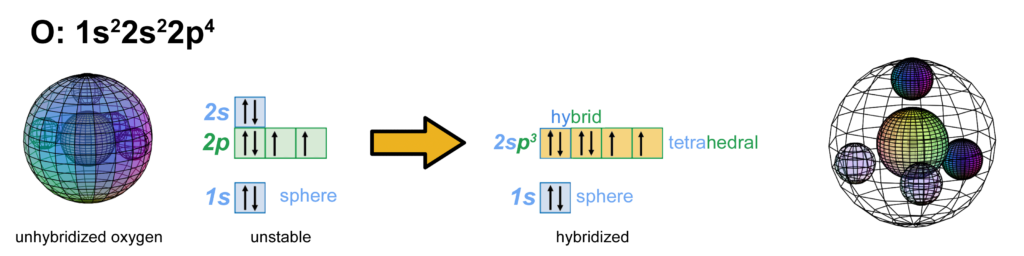
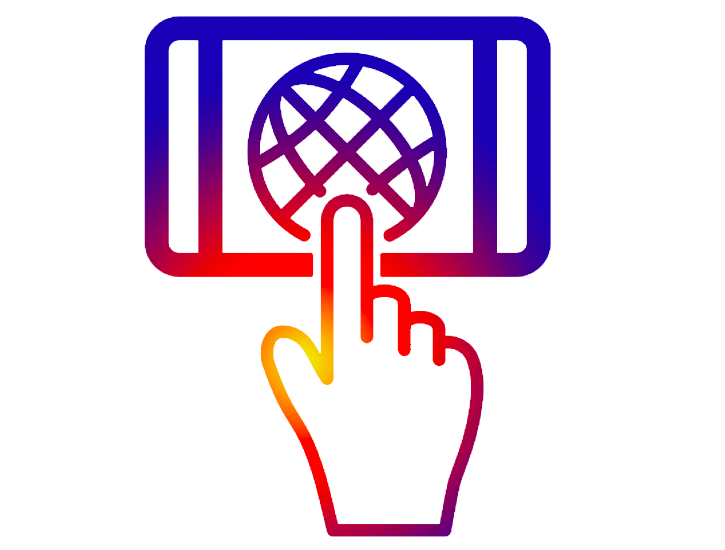
NOTE: The small spheres in the image above simply indicate the directions of maximum electron density. The 2nd shell hybrid orbitals themselves will be more like spherical tetrahedra that fill the volume within their shell with electron density. It will be highest at the center of the face of each orbital (as in the traditional sp3 lobe shapes) and will decrease toward the nodal regions between orbitals — as wave structures usually do — where electron density will be lowest (though not zero).
NOTE ALSO: Even though it is often useful to talk about these orbitals as separate, they are all — the entire atom is — part of a single, coherent, harmonic, resonant, phase-locked, spherically-symmetrical quantum wave state, and it is all electromagnetic at the root-energy level. Orbitals and their ‘boundaries’ can be seen as nothing more than nodes and antinodes in this harmonic wave structure.
In the case of oxygen, the orbitals containing the two ‘di-electron’ lone pairs (dark blue) will each occupy slightly more volume than the two containing the unpaired electrons.
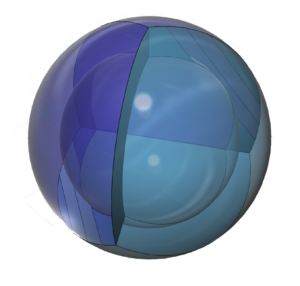
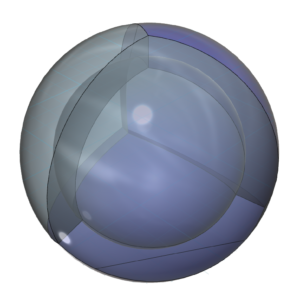
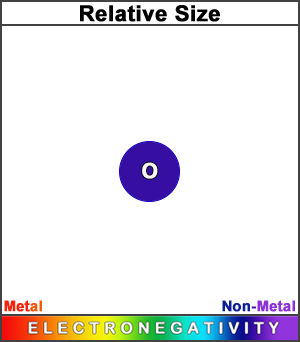
Oxygen has a smaller atomic radius than nitrogen. (This square represents the size of the largest atom).
Both its decrease in size and its increase in electronegativity (across the period) result from its larger effective nuclear charge. Its resulting strong unwillingness to give up an electron makes it very strongly non-metallic.
Bonding & ion formation
As a result of its strong effective nuclear charge, oxygen is very keen to obtain two extra electrons to fill its 2nd shell and achieve maximum stability. That would be the same electron configuration as the 2s22p6 noble gas configuration of neon — a multi-di-electron state with two concentric full shells. One way oxygen can do this is by making two covalent bonds. (See examples below.) Oxygen is the second most electronegative element (after fluorine), and it can therefore be very reactive as it strongly attracts electrons to itself.
Oxygen can also fill its 2nd shell by gaining two electrons in an ionic interaction (with a metal atom). That is why oxygen forms a 2– ionic state. The negative ion is much larger than the neutral atom because electrons now outnumber protons by two. This results in a much lower effective nuclear charge — a lower average attraction by the nucleus on each electron.
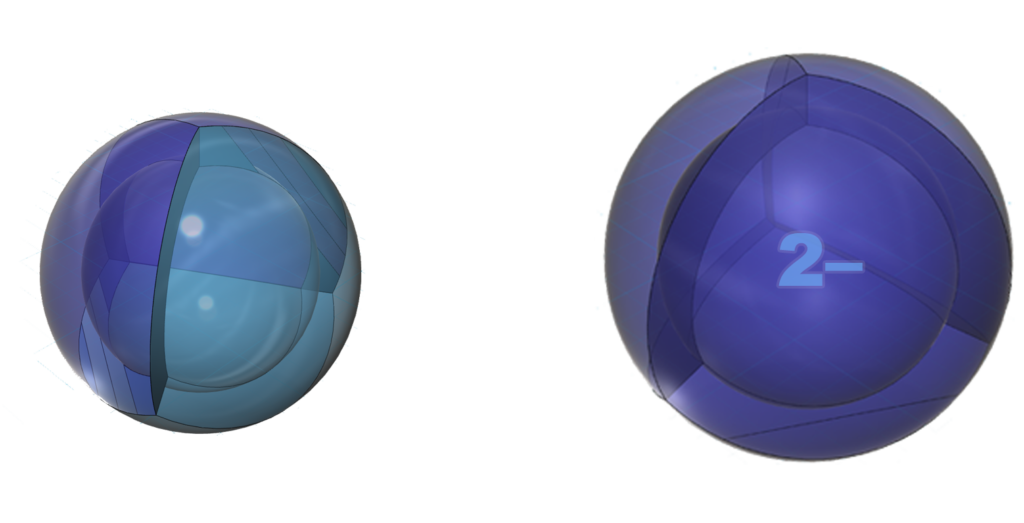
Water (H2O)
When oxygen gains two electrons via covalent bonding with two hydrogen atoms, the water (H2O) molecule is formed. Its asymmetrical structure, and the fact that oxygen pulls electrons more strongly than hydrogen, gives it very important properties. The most significant is that it creates an electron imbalance which makes the side of the molecule where the hydrogen atoms attach slightly positive (𝛿+) and the opposite (oxygen) side slightly negative (𝛿–). This polarity causes water molecules to stick to each other and to certain substances rather effectively. This makes it harder to change water’s temperature, causing oceans and lakes to moderate local climates. It makes ice less dense than water, causing ice to float, ensuring that aquatic species survive the winter. It allows a great variety of substances (like NaCl) to dissolve in water, and it allows others (like oils) to resist water and form cellular structures within a water environment. It is truly a remarkable molecule.
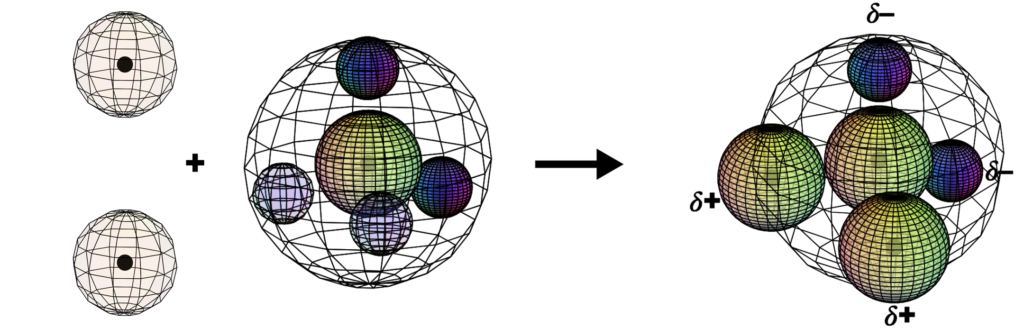
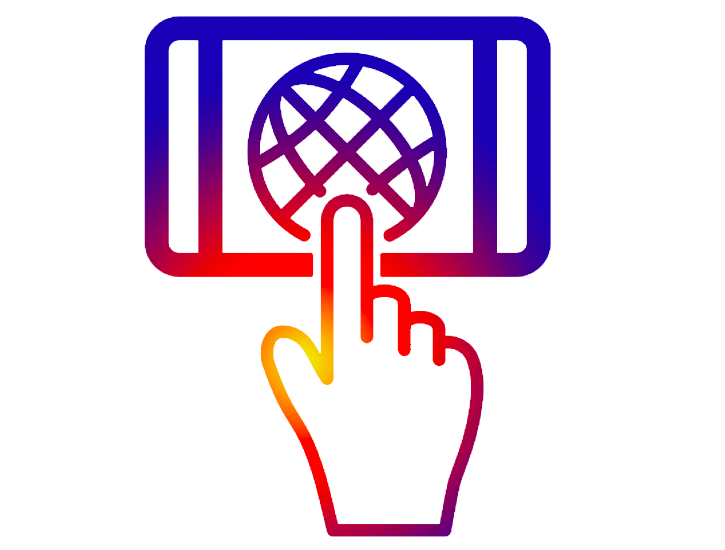
Below is another visualization of the formation of water from the point of view of electronegativity. The electrons in a bond will always be pulled more strongly towards the atom that is closer to the violet end of the electronegativity spectrum. This clarifies precisely why the water molecule is polar. (The bright yellow regions represent the two lone pairs of electrons in oxygen’s outer shell, making it quite electron-rich, and therefore a very attractive proposition for any positive ions in the vicinity. And vice versa on the opposite end. This is why ionic salts dissolve so readily in water.)
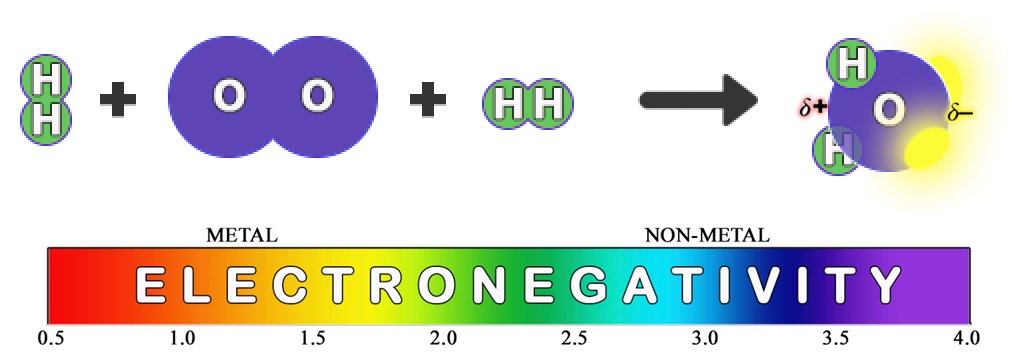
Given the small size of the oxygen atom, the water molecule is also rather small. Unlike atoms, which only tend to gain or lose electrons, the water molecule expresses both positive and negative polarity. The combination of these two facts illuminates why so many crystals in nature contain water molecules within their matrices. It makes for a very versatile structural element in an environment of positive and negative ions.
Combustion Reactions
Oxygen has a high electronegativity, which means that oxygen atoms pull electrons strongly toward themselves. This causes them to react and bond (share electrons) with other atoms in order to gain some of their electron density. It is difficult for an oxygen atom to do that when bonding to another oxygen atom, though, because they are both competing for the shared electrons equally strongly. But when oxygen is near other atoms, which almost all hold their electrons less tightly, the oxygen atoms will prefer to bond with them because they will be able to attract the other atom’s electron density more successfully.
When atoms combine with oxygen, we call the process combustion because it usually gives off so much heat that it manifests fire or an explosion. An example of this reaction occurs when we burn carbon or hydrocarbon compounds in air.
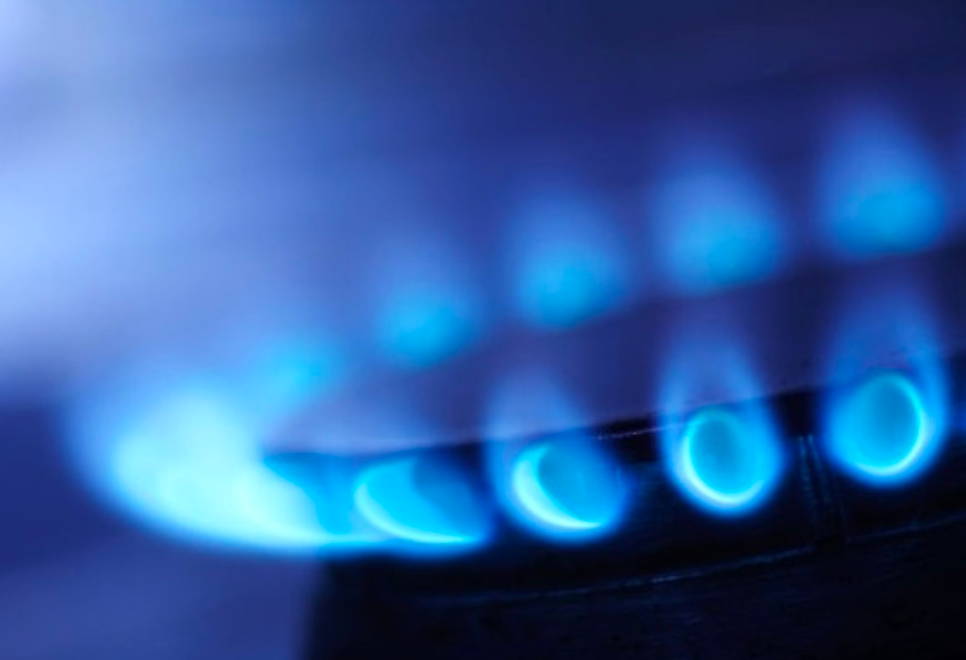
When natural gas (methane CH4) burns (as we see in the image above), oxygen atoms from the oxygen (O2) molecules in the air let go of one another and instead bond to all of the available carbon and hydrogen atoms in the methane because their electrons are easier to attract. Water vapor (H2O) and carbon dioxide (CO2) are formed as a result. The chemical equation for this reaction is:
CH4 + 2O2 —> 2H2O + CO2
Other common combustion reactions occur in the formation of water (H2O) from hydrogen (H2) and oxygen (O2) and the combustion of octane (C8H18) in (the soon-to-be-obsolete) gasoline car engines.
Dioxygen (O2)
The dioxygen (O2) molecule (usually just called “oxygen”) has a surprisingly strong paramagnetism, which means it is strongly attracted into a magnetic field (as shown in the video below). This effect implies that there must be unpaired electrons in the molecule. Unpaired electrons can align themselves with an external magnetic field, and this causes them to be attracted into it. However, the oxygen molecule seems, at first glance, to have no unpaired electrons, so its strong observed paramagnetism needs to be explained via a slightly different orbital theory (see below).
The strength of this magnetic effect is called magnetic susceptibility (χm). By way of perspective, oxygen gas (O2(g)) has a magnetic susceptibility of χm=3,449, which is stronger than the rare earth metal cerium (χm=2,450). Liquid oxygen (O2(l)) has χm=7,699, which is stronger than the rare earth metal neodymium (χm=5,628) — which has four unpaired f-electrons. Furthermore, oxygen gas has more than six times the paramagnetism of manganese (χm=529), which has five unpaired d-electrons.
The Molecular Orbital Theory attributes the paramagnetism of dioxygen (O2) to the presence of two unpaired electrons in anti-bonding molecular orbitals (see Triplet Oxygen). An alternate (and at this point speculative) explanation for this phenomenon may emerge from a sub-quantum mechanical approach, in which a set of quantum electron interactions unique to the oxygen molecule gives rise to a stronger than expected magnetic susceptibility (χm) value. (Details of this model will be shared in due course. Watch the Quicycle Journal for updates.)
Ozone (O3)
A different naturally occurring form (or allotrope) of oxygen is ozone (O3), which consists of three oxygen atoms bonded to each other in a bent shape.
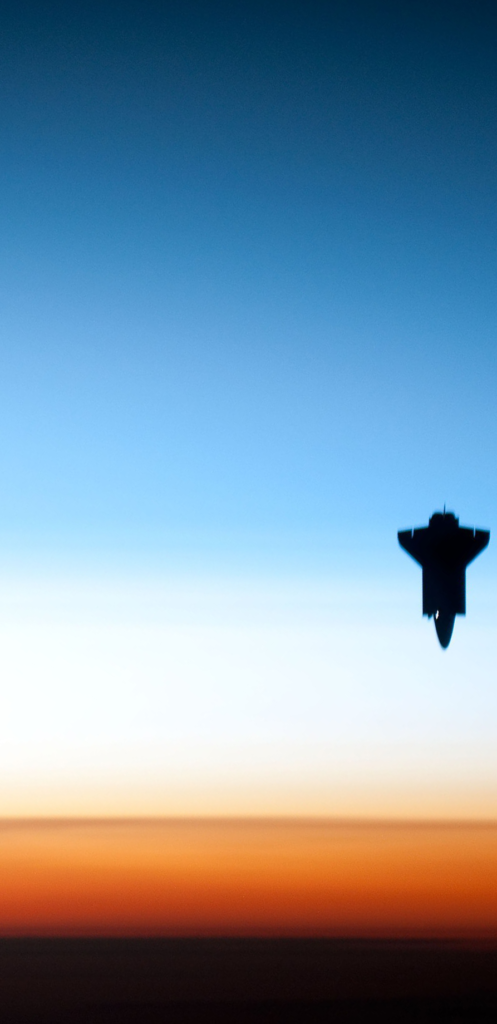
Ozone is formed in the stratosphere when incoming ultraviolet light hits oxygen molecules, and ozone is also broken back down into oxygen when it is hit by ultraviolet light. The ozone layer therefore protects us from harmful radiation by acting as a UV shield in two mutually-reinforcing ways.
Ozone is a molecule that demonstrates an interesting electron state known as resonance. Resonance occurs when the electrons have more than one way to make the same structure, though the structure is not symmetrical overall.
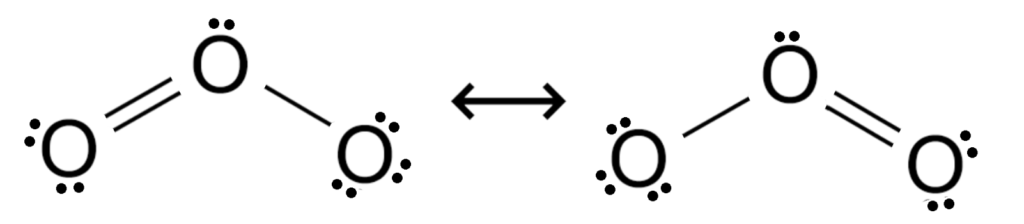
Both versions of this structure feature a single bond, a double bond, and 6 di-electrons. They are exact mirror images of one another, and the traditional understanding of this in chemistry is that the actual structure is some form of average between these two structures. Since that is difficult to depict, we show both alternatives and we imagine the resulting average.
If the Lewis Dot Structure is extended in order to incorporate the sub-quantum mechanical interactions between electrons, especially those related to spin, a single symmetrical resonance state for ozone might looks like this:
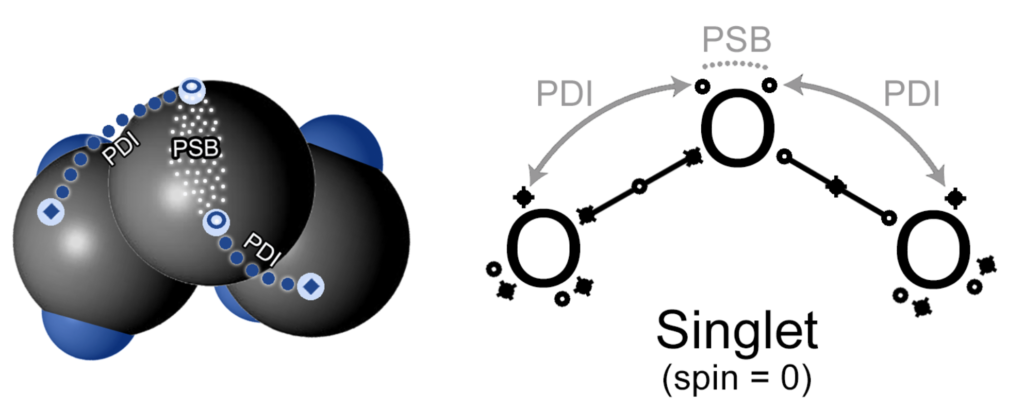
In the diagram above: circles (o) versus diamonds (♦) represent spin-up versus spin-down electrons; each resonance bond thus contains 3 electrons; PSB & PDI are two forms of spin bonding that spin-stabilize otherwise unpaired electrons [ref 1 and 2]. This yields an overall symmetrical and spin=0 (singlet) state for the molecule’s ground state.
RETURN to the Periodic Table